Biochemistry
THE CHEMISTRY OF biological LIFE
![]() |
Biochemistry is the study of the chemistry of life, a bridge between biology and chemistry that studies how complex chemical reactions give rise to life.
It is a hybrid branch of chemistry which specialises in the chemical processes in living organisms. This article only discusses terrestrial biochemistry (carbon- and water-based), as all the life forms we know are on Earth. Since life forms alive today are believed to have descended from the same common ancestor, they naturally have similar biochemistries, even for matters which would appear to be essentially arbitrary, such as the genetic code or handedness of various biomolecules. It is unknown whether alternate biochemistries are possible or practical.
Biochemistry is the study of the structure and function of cellular components, such as proteins, carbohydrates, lipids, nucleic acids, and other biomolecules. Chemical biology aims to answer many questions arising from biochemistry by using tools developed within synthetic chemistry.
Although there is a vast number of different biomolecules, they tend to be composed of the same repeating subunits (called monomers), in different orders. Each class of biomolecules has a different set of subunits. Recently, biochemistry has focused more specifically on the chemistry of enzyme-catalyzed reactions, and on the properties of proteins.
The biochemistry of cell metabolism and the endocrine system has been extensively described. Other areas of biochemistry include the genetic code (DNA, RNA), protein synthesis, cell membrane transport, and signal transduction.
Contents |
Development of biochemistry
Originally, it was generally believed that life was not subject to the laws of science the way nonlife was. It was thought that only living beings could produce the molecules of life (from other, previously existing biomolecules). Then, in 1828, Friedrich Wöhler published a paper about the synthesis of urea, proving that organic compounds can be created artificially. The dawn of biochemistry may have been the discovery of the first enzyme, diastase, in 1833 by Anselme Payen. It is generally accepted that the term biochemistry was coined in 1903 by Carl Neuber, a German chemist. Since then, biochemistry has advanced, especially since the mid-20th century, with the development of new techniques such as chromatography, X-ray diffraction, NMR, radioisotopic labelling, electron microscopy and molecular dynamics simulations. These techniques allowed for the discovery and detailed analysis of many molecules and metabolic pathways of the cell, such as glycolysis and the Krebs cycle (citric acid cycle).
Today, the findings of biochemistry are used in many areas, from genetics to molecular biology and from agriculture to medicine. The first application of biochemistry was probably the making of bread using yeast, about 5000 years ago.
Carbohydrates
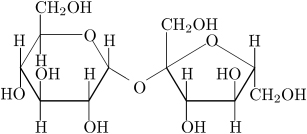
Sucrose: ordinary table sugar and probably the most familiar carbohydrate
The function of carbohydrates includes energy storage and providing structure. Sugars are carbohydrates, although there are carbohydrates that are not sugars. There are more carbohydrates on Earth than any other type of biomolecule. The simplest type of carbohydrate is a monosaccharide, which among other properties contains carbon, hydrogen, and oxygen in a ratio 1:2:1 (generalized formula CnH2nOn, where n is at least 3). Glucose, one of the most important carboyhydrates, is an example of a monosaccharide. So is fructose, the sugar that gives fruits their sweet taste.
Two monosaccharides can be joined together using dehydration synthesis, in which a hydrogen atom is removed from the end of one molecule and a hydroxyl group (—OH) is removed from the other; the remaining residues are then attached at the sites from which the atoms were removed. The H—OH or H2O is then released as a molecule of water, hence the term dehydration. The new molecule, consisting of two monosaccharides, is called a disaccharide and is conjoined together by a glycosidic or ether bond. The reverse reaction can also occur, using a molecule of water to split up a disaccharide and break the glycosidic bond; this is termed hydrolysis. The most well-known disaccharide is sucrose, ordinary sugar (in scientific contexts, called table sugar or cane sugar to differentiate it from other sugars). Sucrose consists of a glucose molecule and a fructose molecule joined together. Another important disaccharide is lactose, consisting of a glucose molecule and a galactose molecule. As most humans age, the production of lactase, the enzyme that hydrolyzes lactose back into glucose and galactose, typically decreases. This results in lactase deficiency, also called lactose intolerance.
When a few (around three to six) monosaccharides are joined together, it is called an oligosaccharide (oligo- meaning "few"). These molecules tend to be used as markers and signals, as well as having some other uses.
Many monosaccharides joined together make a polysaccharide. They can be joined together in one long linear chain, or they may be branched. Two of the most common polysaccharides are cellulose and glycogen, both consisting of repeating glucose monomers. Cellulose is made by plants and is an important structural component of their cell walls. Humans can neither manufacture nor digest it. Glycogen, on the other hand, is an animal carbohydrate; humans use it as a form of energy storage.
Image:Glycolysis10steps.gif Glucose is the major energy source in most life forms; a number of catabolic pathways converge on glucose. For instance, polysaccharides are broken down into their monomers (glycogen phosphorylase removes glucose residues from glycogen). Disaccharides like lactose or sucrose are cleaved into their two component monosaccharides. Glucose is metabolized by a very important and ancient ten-step pathway called glycolysis, the net result of which is to break down one molecule of glucose into two molecules of pyruvate; this also produces a net two molecules of ATP, the energy currency of cells, along with two reducing equivalents in the form of converting NAD to NADH. This does not require oxygen; if no oxygen is available (or the cell cannot use oxygen), the NAD is restored by converting the pyruvate to lactate (in humans, for instance) or to ethanol in yeast. Other monosaccharides like galactose and fructose can be converted into intermediates of the glycolytic pathway. In aerobic cells with sufficient oxygen, like most human cells, the pyruvate can be further metabolized. It is irreversibly converted to acetyl-CoA, giving off one carbon atom as the waste product carbon dioxide, generating another molecule of ATP, and generating another reducing equivalent as NADH. The two molecules acetyl-CoA (from one molecule of glucose) then enter the citric acid cycle, producing two more molecules of ATP, six more NADH molecules and two of a related molecule FADH2, and releasing the remaining carbon atoms as carbon dioxide. The reduced NADH and FADH2 then enter the electron transport system, where the electrons are transferred to a molecule of oxygen, producing water, and the original NAD+ and FAD are regenerated. This is why humans breath in oxygen and breath out carbon dioxide. The energy in transferring the electrons from high-energy states in NADH and FADH2 is used to generate an additional 28 molecules of ATP (only two had been produced in glycolysis), for a total of 32 molecules of ATP. It is clear that using oxygen to completely oxidize glucose provides an organism with far greater energy, and it is why complex life appeared only after Earth's atmosphere accumulated large amounts of oxygen.
In vertebrates, vigorously contracting skeletal muscle (during weightlifting or sprinting, for example) does not receive enough oxygen to meet the energy demand, and so it shifts to anaerobic metabolism, converting glucose to lactate (lactic acid). The liver can regenerate the glucose, using a process called gluconeogenesis. This process is not quite the opposite of glycolysis, and actually requires three times the amount of energy gained from glycolysis (six molecules of ATP are used, compared to the two gained in glycolysis). Analogous to the above reactions, the glucose produced can then undergo glycolysis in tissues that need energy, be stored as glycogen (or starch in plants), or be converted to other monosaccharides or joined into di- or oligosaccharides.
Proteins
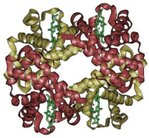
A schematic of hemoglobin. The ribbon parts represent the protein globin; the four green parts are the heme groups.
Like carbohydrates, some proteins perform largely structural roles. For instance, movements of the proteins actin and myosin ultimately are responsible for the contraction of skeletal muscle. One property many proteins have is that they specifically bind to a certain molecule or class of molecules—they may be extremely selective in what they bind. Antibodies are an example of proteins that attach to one specific type of molecule. In fact, the enzyme-linked immunosorbent assay (ELISA), which uses antibodies, is currently one of the most sensitive tests modern medicine uses to detect various biomolecules. Probably the most important proteins, however, are the enzymes. These amazing molecules recognize specific reactant molecules called substrates; they then catalyze the reaction between them. By lowering the activation energy, the enzyme speeds up that reaction by a rate of 1011 or more: a reaction that would normally take over 3,000 years to complete spontaneously might take less than a second with an enzyme. The enzyme itself is not used up in the process, and is free to catalyze the same reaction with a new set of substrates. Using various modifiers, the activity of the enzyme can be regulated, enabling control of the biochemistry of the cell as a whole.
In essence, proteins are chains of amino acids. An amino acid consists of a carbon atom bound to four groups. One is an amino group, —NH2, and one is a carboxylic acid group, —COOH (although these exist as —NH3+ and —COO− under physiologic conditions). The third is a simple hydrogen atom. The fourth is commonly denoted "—R" and is different for each amino acid. There are twenty standard amino acids. Some of these have functions by themselves or in a modified form; for instance, glutamate functions as an important neurotransmitter.
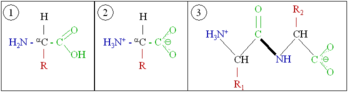
Generic amino acids (1) in neutral form, (2) as they exist physiologically, and (3) joined together as a dipeptide.
Amino acids can be joined together via a peptide bond. In this dehydration synthesis, a water molecule is removed and the peptide bond connects the nitrogen of one amino acid's amino group to the carbon of the other's carboxylic acid group. The resulting molecule is called a dipeptide, and short stretches of amino acids (usually, fewer than around thirty) are called peptides or polypeptides. Longer stretches merit the title proteins. As an example, the imporant blood serum protein albumin contains 585 amino acid residues.
The structure of proteins is traditionally described in a hierarchy of four levels. The primary structure of a protein simply consists of its linear sequence of amino acids; for instance, "alanine-glycine-tryptophan-serine-glutamate-asparagine-glycine-lysine-...". Secondary structure is concerned with local morphology. Some combinations of amino acids will tend to curl up in a coil called an α-helix; some of these can be seen in the hemoglobin schematic above. Tertiary structure is the entire three-dimensional shape of the protein. This shape is determined by the sequence of amino acids. In fact, a single change can change the entire structure. The β chain of hemoglobin contains 146 amino acid residues; substitution of the glutamate residue at position 6 with a valine residue changes the behavior of hemoglobin so much that it results in sickle-cell disease. Finally quaternary structure is concerned with the structure of a protein with multiple peptide subunits, like hemoglobin with its four subunits. Not all proteins have more than one subunit.
Ingested proteins are usually broken up into single amino acids or dipeptides in the small intestine, and then absorbed. They can then be joined together to make new proteins. Intermediate products of glycolysis, the citric acid cycle, and the pentose phosphate pathway can be used to make all twenty amino acids, and most bacteria and plants possess all the necessary enzymes to synthesize them. Humans and other mammals, however, can only synthesize half of them. They cannot synthesize isoleucine, leucine, lysine, methionine, phenylalanine, threonine, tryptophan, and valine. These are the essential amino acids, since it is essential to ingest them. Mammals do possess the enzymes to synthesize alanine, asparagine, aspartate, cysteine, glutamate, glutamine, glycine, proline, serine, and tyrosine, the nonessential amino acids. While they can synthesize arginine and histidine, they cannot produce it in sufficient amounts for young, growing animals, and so these are often considered essential amino acids.
If the amino group is removed from an amino acid, it leaves behind a carbon skeleton called an α-keto acid. Enzymes called transaminases can easily transfer the amino group from one amino acid (making it an α-keto acid) to another α-keto acid (making it an amino acid). This is important in the biosynthesis of amino acids, as for many of the pathways, intermediates from other biochemical pathways are converted to the α-keto acid skeleton, and then an amino group is added, often via transamination. The amino acids may then be linked together to make a protein.
A similar process is used to break down proteins. It is first hydrolyzed into its component amino acids. Free ammonia (NH3, existing as the ammonium ion NH4+) in blood) is toxic to life forms. A suitable method for excreting it must therefore exist. Different strategies have evolved in different animals, depending on the animals' needs. Unicellular organisms, of course, simply release the ammonia into the environment. Similarly, bony fish can release the ammonia into the water where it is quickly diluted. In general, mammals convert the ammonia into urea, via the urea cycle.
Lipids
The term lipid comprises a diverse range of molecules and to some extent is a catchall for relatively water-insoluble or nonpolar compounds of biological origin, including waxes, fatty acids, fatty-acid derived phospholipids, sphingolipids, glycolipids and terpenoids, such as retinoids and steroids. Some lipids are linear aliphatic molecules, while others have ring structures. Some are aromatic, while others are not. Some are flexible, while others are rigid.
Most lipids have some polar character in addition to being largely nonpolar. Generally, the bulk of their structure is nonpolar or hydrophobic ("water-fearing"), meaning that it does not interact well with polar solvents like water. Another part of their structure is polar or hydrophilic ("water-loving") and will tend to associate with polar solvents like water. This makes them amphiphilic molecules (having both hydrophobic and hydrophilic portions). In the case of cholesterol, the polar group is a mere -OH (hydroxyl or alcohol). In the case of phospholipids, the polar groups are considerably larger and more polar, as described below.
Nucleic acids
A nucleic acid is a complex, high-molecular-weight biochemical macromolecule composed of nucleotide chains that convey genetic information. The most common nucleic acids are deoxyribonucleic acid (DNA) and ribonucleic acid (RNA). Nucleic acids are found in all living cells and viruses.
Nucleic acid, so called because of its prevalence in cellular nuclei, is the generic name of family of biopolymers. The monomers are called nucleotides, and each consists of three components: a nitrogenous heterocyclic base (either a purine or a pyrimidine), a pentose sugar, and a phosphate group. Different nucleic acid types differ in the specific sugar found in their chain (e.g. DNA or deoxyribonucleic acid contains 2-deoxyriboses). Also, the nitrogenous bases possible in the two nucleic acids are different: adenine, cytosine, and guanine are possible in both RNA and DNA, while thymine is possible only in DNA and uracil is possible only in RNA.
Relationship to other "molecular-scale" biological sciences
Schematic relationship between biochemistry, genetics and molecular biology
Researchers in biochemistry use specific techniques native to biochemistry, but increasingly combine these with techniques and ideas from genetics, molecular biology and biophysics. There is not a hard-line between these disciplines as there once was. The following figure is a schematic that depicts one possible view of the relationship between the fields:
- Biochemistry is the study of the chemical substances and vital processes occurring in living organisms.
- Genetics is the study of the effect of genetic differences on organisms. Often this can be inferred by the absence of a normal component (e.g. one gene). The study of "mutants" – organisms which lack one or more functional components with respect to the so-called "wild type" or normal phenotype. Genetic interactions (epistasis) can often confound simple interpretations of such "knock-out" studies.
- Molecular biology is the study of molecular underpinnings of the process of replication, transcription and translation of the genetic material. The central dogma of molecular biology where genetic material is transcribed into RNA and then translated into protein, despite being an oversimplified picture of molecular biology, still provides a good starting point for understanding the field. This picture, however, is undergoing revision in light of emerging novel roles for RNA.
- Chemical Biology seeks to develop new tools based on small molecules that allow minimal perturbation of biological systems while providing detailed information about their function. Further, chemical biology employs biological systems to create non-natural hybrids between biomolecules and synthetic devices (for example emptied viral capsids that can deliver gene therapy or drug molecules).
RELATED
STUDY